Carotid haemodynamics during sympathetic nervous system stimulation via handgrip and cold pressor testing in young healthy subjects: A feasibility study
- DOI
- 10.1016/j.artres.2014.08.001How to use a DOI?
- Keywords
- Biosignals; Medical imaging; Arterial stiffness; Baroreflex; Cardiovascular control
- Abstract
Introduction: Assessing arterial and haemodynamic properties in response to a physiological perturbation might provide additional information on an individual’s “vascular health”. The aim of this study was to assess the feasibility of measuring changes in carotid stiffness and haemodynamics in response to sympathetic nervous system stressors, such as the Hand Grip Test (HGT) and the Cold Pressor Test (CPT).
Methods: A non-invasive protocol, consisting of two HGT’s at 30% and 40% of maximal voluntary contraction and a CPT, was performed in 12 young healthy volunteers (6 males/6 females). Measurements included continuous finger blood pressure recordings (NexFin; non-dominant hand) and ultrasound measurement of common carotid diameter distension and flow velocity at discrete moments in time during the protocol (GE Vivid 7 system). Carotid distension (ΔD/D), local wave speed using the waterhammer equation (PWVWH) and reflection magnitude (RM; ratio of backward to forward diameter wave) were derived from the data.
Results: Consistent with the overall increase in blood pressure, carotid diameter increased while ΔD/D decreased. PWVWH and RM showed high variability during both HGT’s and CPT.
Conclusion: It is feasible to monitor the carotid haemodynamic response to selected sympathetic nervous system stimulus. Moreover, the analysis showed supporting evidence of specific physiological response to regulate adequately each stress stimulus.
- Copyright
- © 2014 Association for Research into Arterial Structure and Physiology. Published by Elsevier B.V. All rights reserved.
- Open Access
- This is an open access article distributed under the CC BY-NC license.
Introduction
Common functional measurements to assess the cardiovascular system (cardiac systolic and diastolic function or arterial stiffness, among others) are typically performed during resting conditions. However, in real life, humans are active and there is an intrinsic variability in the cardiovascular status that is, probably, necessary to take into account when interpreting the outcome of these type of tests. This variability is related to the Autonomic Nervous System (ANS), which regulates the visceral functions of the body and helps to control arterial blood pressure, sweating, body temperature, and many other activities. One of the most striking characteristics of the autonomic nervous system is the rapidity and intensity with which it can change visceral functions.1 Thus, the nervous system, acting through the autonomic nerve fibres, can exert rapid and effective control of many if not most of the internal functions of the body. The signals are transmitted to the body through two major subdivisions called the sympathetic and parasympathetic systems.1 Therefore, assessment of the ANS can provide additional information of the cardiovascular control and general state of a subject. This assessment can be done through the use of sympathetic nervous stressors such as the hand grip test (HGT) or the cold pressor test (CPT).
Physical activity, for example isometric exercise (HGT), produces intensity-dependent increases in arterial blood pressure that are mediated by central signals arising from higher brain centres (i.e., central command) and by peripheral feedback from skeletal muscle (i.e., exercise pressor reflex; EPR) with further modulation provided by the arterial baroreflex2 (see Fig. 1A). The arterial baroreflex is a critical cardiovascular reflex that provides a continuous buffering of acute fluctuations of arterial blood pressure (BP) controlling two of the major variables that determine it: cardiac output and total peripheral resistance.5 HGT produces simultaneous increases in BP and heart rate, which are not (totally) blunted by the arterial baroreflex. This led to early speculation that baroreflexes are altered in some fashion to allow this deviation from normal baroreflex physiology to occur.7 The current understanding of the mechanisms for baroreflex resetting during exercise is that the feed forward mechanism of central command is probably the primary regulator of baroreflex resetting, while the negative feedback mechanism of the EPR is more of a modulator of resetting8 (see Fig. 1C).

A) Isometric exercise: It produces intensity-dependent increases in arterial blood pressure (BP) that are mediated by central signals arising from higher brain centres (i.e., central command) and by peripheral feedback from skeletal muscle (i.e., exercise pressor reflex; EPR) with further modulation provided by the arterial baroreflex.2 Classically, the central command is defined as a feed forward mechanism involving parallel activation of motor and cardiovascular centres.3 In the EPR or the “muscle metaboreflex”, neural signals arising from stimulation of chemosensitive receptors in the contracting muscles would activate reflexly the cardiovascular control areas in the medulla.4 The arterial baroreflex is a critical cardiovascular reflex that provides a continuous buffering of acute fluctuations of arterial BP controlling the two variables that determine it: cardiac output and total peripheral resistance.5 B) Cold pressor test: In most healthy human subjects, cutaneous application of ice water (i.e., CPT), increases arterial pressure, heart rate, and vascular resistance. Cold nociceptors in the skin conduct afferent signals by unmyelinated C-fibres, and the pathway may involve central vasomotor centres that serve to regulate muscle sympathetic nerve activity (MSNA). Thus, CPT is performed to asses sympathetic neural control of the peripheral and coronary circulations in humans.6 C) Baroreflex stimulus–response curve and resetting during exercise, where the centring point is the point at which there is an equal depressor and pressor response to a given change in blood pressure, the operating point is the prestimulus blood pressure, threshold is the point where no further increases in heart rate are elicited by further reductions in blood pressure, and saturation is the point where no further decreases in heart rate are elicited by further increases in blood pressure. The relocation of the operating point away from the centring point and closer to the threshold of the stimulus–response curve with increasing exercise intensity modifies the baroreflex in a more optimal condition to counter hypertensive stimuli.3
Cold pressor tests (CPT) are often performed in the clinical evaluation of autonomic disorders to ensure the integrity of central vasomotor processes and their efferent pathways. The test elicits remarkable increases in arterial pressure and muscle sympathetic nerve activity (MSNA) with no significant changes in heart rate.9 In most healthy human subjects, cold nociceptors in the skin conduct afferent signals that serve to regulate MSNA9 thus CPT is performed to asses sympathetic neural control of the peripheral and coronary circulations in humans6 (see Fig. 1B). Besides, CPT is suggested to be a method that mimics the effects of chronic pain conditions effectively because of its unpleasantness, and it has excellent reliability and validity hence fast and efficient evaluations of pain treatments are made possible in healthy participants, making this technique a useful and less expensive prerequisite to clinical studies.10
Both described stressors have distinct neural paths and receptors9 (see Fig. 1) and consequently, a differential response to each stimulus should be measurable selecting an adequate anatomical target and appropriate parameters. We hypothesize that the functional assessment of carotid arterial properties and haemodynamics would be a good candidate for such investigation due to its relation with the baroreflex.
The aim of this study was therefore to assess the feasibility of non-invasively monitoring carotid hemodynamics in response to sympathetic nervous system stressors. Ultrasound was used to assess blood flow velocity and carotid diameter distension waveforms throughout the protocol, with subsequent derivation of functional parameters related to the tonus (stiffness – local carotid pulse wave velocity) of the carotid artery and the downstream vascular bed (the reflection magnitude).
Methods
Subjects
Measurements were performed on twelve young (presumably) healthy volunteers (six males, six females). Subjects were instructed to refrain from eating and ingesting beverages containing caffeine and alcohol for at least 2 h before testing. Volunteers were studied in supine position in a quiet, lightly dimmed room (temperature 25–28 °C).
Protocol instrumentation and set up
Measurements included continuous haemodynamic recordings using a finger cuff with an optical plethysmograph (Nexfin, BMEYE B.V., Amsterdam, The Netherlands) and provided systolic blood pressure (SBP), diastolic blood pressure (DBP), the blood pressure (BP) waveform and heart rate (HR). Ultrasound (US) measurements of the carotid artery were done at discrete moments during the protocol (Vivid7, GE-Vingmed Ultrasound AS, Horten, Norway) and consisted of 1) diameter distension measurements using a “wall tracking” application11 and 2) Pulsed Wave Doppler (PW).
Figure 2 shows the general set up during the protocol: analogue outputs of the Nexfin monitor (number 1) were connected to an external acquisition device (number 2, SC-2345, National instruments, Austin (Texas), United States), which sents the signals to a PC (number 3). During measurements, a holder was used to fix the US probe (number 6) on the carotid artery (volunteer’s dominant hand side). In Fig. 2A, the set up for the hand grip test (HGT) is shown. The hand grip device (number 7, Precision dynamometer G200, Biometrics Ltd, Newport, United Kingdom) was connected to the external PC (number 3) to provide a visual feedback to the volunteer during the test. In Fig. 2B, the cold pressor test (CPT) setup is shown. During the test, the subject immersed the dominant hand in cold water (number 7) for about 3 min.

Protocol implementation
Before the start of the protocol, basic anthropometric measurements were performed while a general explanation of the procedures was given to each volunteer. Then, the subject was asked to lay down and to perform three brief maximal voluntary contractions with her/his dominant hand using the hand grip device. The highest value obtained was selected as the reference value and defined as the maximal voluntary contraction (MVC). Following, to achieve a haemodynamic steady state in each volunteer, there was an initial resting period of at least 20 min in supine position.
Hand grip test (isometric exercise)
The first test performed was an HGT at 30% of the MVC (HGT30%) followed by a resting period. Taking into account the time limitations and values used in literature,9,12–14 it was decided that a resting time period of 10 min in between tests was adequate. Next, a second HGT was carried out at 40% of the MVC (HGT40%).
For both tests, US measurements were obtained at baseline, during the test and upon recovery. The time span of the test period was 2 min for HGT30% and 90 s for HGT40%, during which time subjects were provided with visual feedback of their contraction force production. In all cases, US measurements were performed only after one minute of the onset of test and recovery periods.
Cold pressor test
With a resting period in between HGT40% and CPT of at least 10 min and after baseline measurements were performed, the subjects immersed their dominant hand up to the wrist into a cold water bath (0–5 °C) for a period of ∼3 min. Two US measurements were performed: during the first half of the test (between the onset and 90 s) and during the final part of the test (between 90 s and the end). This second measurement was eventually used to perform the analysis of results. This test was performed as fast as possible to avoid discomfort of the volunteers, therefore the timing differed accordingly for each subject. When the test period was over, the last US measurement was obtained after at least 1 min recovery.
A general summary of the protocol is presented in Table 1
Step | Action | ||
---|---|---|---|
MVC | Determination of Maximal Voluntary Contraction | ||
Rest | 20 min (Minimum) | ||
HGT30% | Baseline | 30% MVC (120 s) | Recovery |
Rest | 10 min (Minimum) | ||
HGT40% | Baseline | 40% MVC (90 s) | Recovery |
Rest | 10 min (Minimum) | ||
CPT | Baseline | CPT (180 s) | Recovery |
HGT30% = Hand Grip Test performed at 30% of the MVC, HGT40% = Hand Grip Test performed at 40% of the MVC, CPT = Cold Pressor Test.
Overview of the implemented protocol.
Signal processing
All post processing was done offline thus all signals were saved, stored and labelled for later use. Continuous haemodynamic signals (SBP/DBP/HR/BP wave) were sampled at 200 Hz and saved as ASCII files. These signals were divided into three established periods (baseline, test and recovery). The test period was split as well in two parts, being expected that the reaction to the stressors is more prominent during the second part (see Fig. 3), and therefore this was used for further analysis. Finally, a mean value (and standard deviation) of each period was calculated and used for post processing.

Haemodynamic signal obtained with Nexfin monitor (finger cuff) during HGT40%. Systolic blood pressure (SBP) (Top signal envelop), diastolic blood pressure (DBP) (Bottom signal envelop) and blood pressure wave. Haemodynamic parameters were calculated by the division of the signal in three periods (baseline, test and recovery) to obtain a final value as the mean of each period. Using timing data, the test period was divided in two parts. The second part was used to calculate derived parameters and to perform comparisons against baseline data.
Mean arterial pressure (MAP) was calculated from diastolic and systolic blood pressure values using the equation15:
An RF data based wall-tracking application11 in Matlab was used to obtain a diameter distension waveform (see Fig. 4, top left) from which carotid distension (ΔD/D) was calculated as:
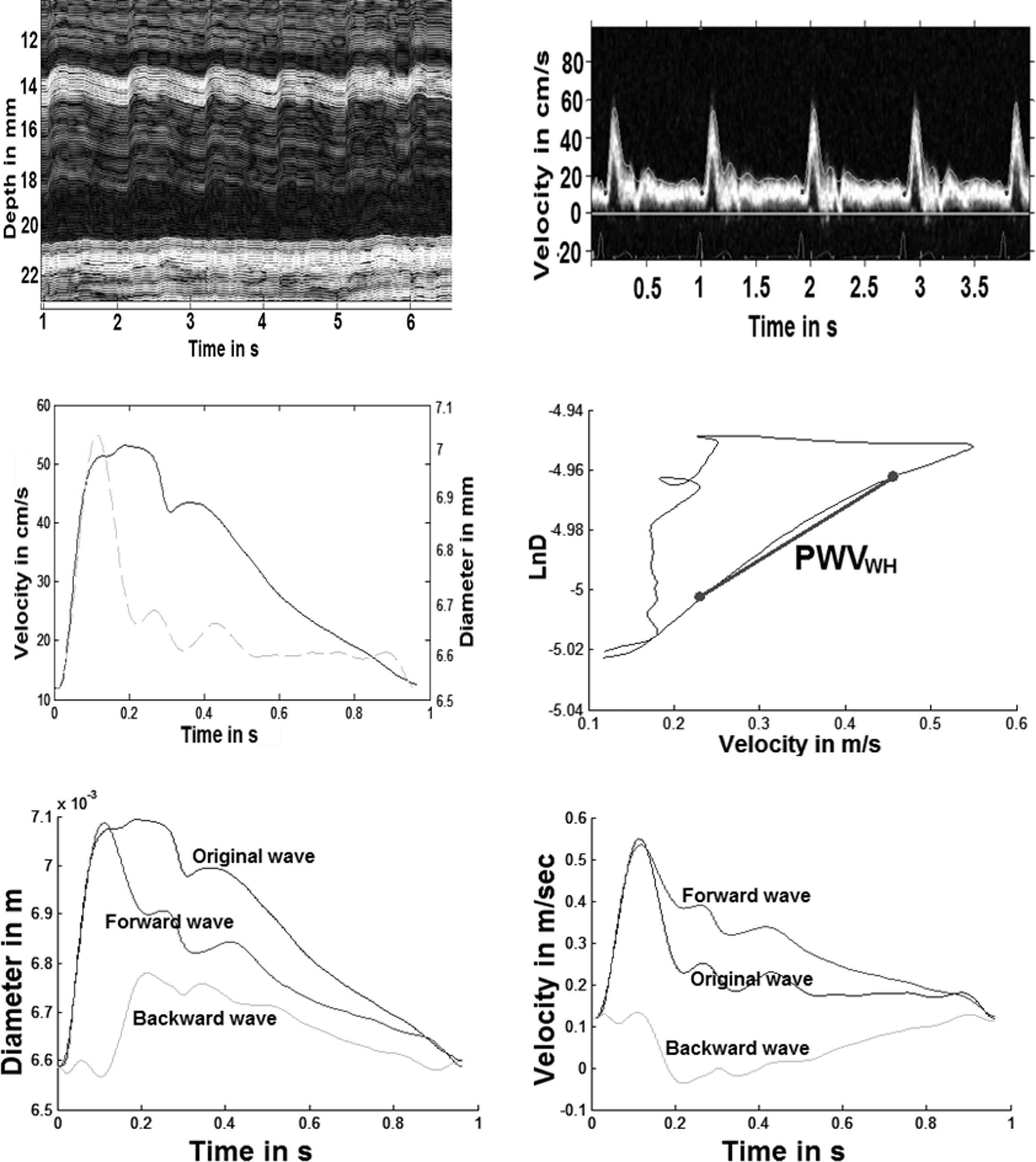
General segmentation and post processing methodology applied to protocol signals. Top left) Manual wall tracked segmentation results using US images of the carotid artery (dominant hand side) during CPT. Top right) PW mode US signal of carotid blood flow velocity and segmentation results: signal envelop was used as velocity curve. The velocity curve was divided in cardiac cycles to obtain an average curve (of at least 3 cardiac cycles) for post processing: each cycle was defined between two consecutive minimal blood flow velocity points before the steep rise in velocity during systole. Mean blood flow velocity was obtained as the average of the values of a velocity curve, while the peak blood flow velocity was the maximal value of the velocity curve. Middle left) Alignment result of the carotid blood flow velocity (dashed line) and diameter distension (solid line) curves using the correlation method.16 Middle right) LnDU-loop built plotting the natural logarithm of the carotid artery diameter distension curve against the blood flow velocity curve. According to the used methodology,17 the slope of the loop’s linear portion indicates the value of the local pulse wave velocity (PWVWH). Bottom) Wave separation17 results of carotid artery: left) diameter distension and right) blood flow velocity curves, indicating the original wave and the derived forward and backward waves.
The envelope of the blood flow velocity waveform was automatically traced from pulse wave (PW) Doppler mode US measurements and divided in individual cardiac cycles, providing information about the flow velocity changes in the carotid artery. At least three cycles were obtained from each image for deriving mean and peak blood flow velocities (see Fig. 4, top right: segmented PW Doppler US mode image. Middle left: carotid blood flow velocity curve (dashed line)).
Carotid pulse wave velocity and reflection magnitude
As carotid diameter distension and blood flow velocity curves are not acquired simultaneously, they had to be aligned in time to perform further processing. We implemented a correlation method16 to automatically align the signals as displayed in Fig. 4 (middle left).
As described by Feng et al,17 the local pulse wave velocity (PWVWH) can be derived from the blood flow velocity curve (U) and the natural logarithm of the diameter distension curve (LnD) via the well-established water hammer equation (3). Plotting LnD against U gives a LnDU-loop, as the one shown in Fig. 4 (middle right), and a linear portion is expected during the early part of systole, when it is most probable that reflected waves do not exist.17 Two points were selected (manually) from the linear portion of the loop to determine the slope of the segment, and therefore, the local PWVWH.
Adapting the water hammer equation to relate changes of diameter (D) and blood flow velocity (U), forward (D+, U+) and backward (D−, U−) waveforms can then be obtained (see Fig. 4 bottom) from which the magnitude of wave reflection can be derived as the ratio of the amplitude of D+ and D−18:
Statistical analysis
Wilcoxon signed rank tests were applied to compare baseline against test and recovery mean values. Statistical significant difference was accepted at a p-value ≤ 0.05. Data are presented as mean± standard deviation.
Results
Measurements were technically feasible in all 12 subjects throughout the complete protocol. However, for 2 subjects, ultrasound data during HGT30% was missing due to storage problems (unrelated to feasibility) while data from one volunteer was missing for HGT40% due to post processing difficulties (see Table 2). The general features of the sample population were: mean age, height and weight of 26 ± 2 years, 173 ± 11 cm and 72 ± 13 kg, respectively.
Baseline | Test | Recovery | ||
---|---|---|---|---|
MAP (mmHg) | HGT30% | 91.00 ± 11.86 | 100.70 ± 13.33b | 94.00 ± 12.22a |
HGT40% | 89.83 ± 6.85 | 101.03 ± 9.05b | 92.49 ± 7.63a | |
CPT | 91.03 ± 6.97 | 102.24 ± 8.59b | 93.96 ± 7.89a | |
SBP (mmHg) | HGT30% | 119.49 ± 16.13 | 131.31 ± 19.71b | 125.01 ± 17.32a |
HGT40% | 118.85 ± 8.12 | 131.13 ± 11.86b | 123.59 ± 9.65a | |
CPT | 119.85 ± 9.23 | 133.53 ± 11.66b | 124.08 ± 9.80a | |
DBP (mmHg) | HGT30% | 72.01 ± 9.47 | 80.30 ± 9.58b | 73.32 ± 9.28 |
HGT40% | 70.48 ± 6.64 | 80.97 ± 7.72b | 71.75 ± 6.74 | |
CPT | 71.81 ± 6.07 | 81.38 ± 7.21b | 73.88 ± 6.94a | |
PP (mmHg) | HGT30% | 47.48 ± 8.44 | 51.00 ± 11.67a | 51.69 ± 9.68b |
HGT40% | 48.38 ± 4.95 | 50.16 ± 6.50a | 51.84 ± 5.13b | |
CPT | 48.04 ± 5.62 | 52.14 ± 7.05a | 50.21 ± 4.59a | |
HR (bpm) | HGT30% | 63.38 ± 6.62 | 69.29 ± 8.76a | 62.82 ± 6.47 |
HGT40% | 61.72 ± 6.43 | 74.80 ± 12.98b | 61.10 ± 5.84 | |
CPT | 59.13 ± 5.56 | 64.25 ± 8.61a | 59.54 ± 6.38 | |
Ds (mm) | HGT30% | 7.45 ± 0.59 | 7.61 ± 0.71c | 7.43 ± 0.60 |
HGT40% | 7.52 ± 0.57 | 7.68 ± 0.65a | 7.45 ± 0.56 | |
CPT | 7.52 ± 0.57 | 7.71 ± 0.64a | 7.46 ± 0.59 | |
ΔD/D | HGT30% | 0.09 ± 0.02 | 0.07 ± 0.02b,c | 0.09 ± 0.02 |
HGT40% | 0.09 ± 0.02 | 0.07 ± 0.02b | 0.09 ± 0.02 | |
CPT | 0.10 ± 0.02 | 0.08 ± 0.02a | 0.10 ± 0.02 | |
L PWVWH (m/s) | HGT30% | 4.07 ± 1.56 | 4.23 ± 3.08c | 3.39 ± 1.51 |
HGT40% | 3.67 ± 1.91 | 4.39 ± 2.73d | 3.44 ± 1.70 | |
CPT | 3.17 ± 1.12 | 3.99 ± 1.06a,d | 4.19 ± 1.64a | |
RM | HGT30% | 0.42 ± 0.08 | 0.43 ± 0.08c | 0.39 ± 0.04 |
HGT40% | 0.42 ± 0.06 | 0.42 ± 0.09d | 0.39 ± 0.04 | |
CPT | 0.42 ± 0.06 | 0.46 ± 0.06d | 0.45 ± 0.06 | |
M BFV (cm/s) | HGT30% | 23.71 ± 5.56 | 22.19 ± 7.04 | 22.79 ± 3.95 |
HGT40% | 21.31 ± 4.13 | 24.18 ± 6.93a | 21.89 ± 5.28 | |
CPT | 22.02 ± 5.38 | 23.88 ± 6.15 | 24.11 ± 6.27 | |
P BFV (cm/s) | HGT30% | 61.56 ± 12.43 | 49.80 ± 12.75a | 59.08 ± 12.03 |
HGT40% | 57.06 ± 11.41 | 53.75 ± 13.32 | 58.27 ± 15.48 | |
CPT | 57.64 ± 14.60 | 57.37 ± 14.98 | 66.83 ± 19.83a |
Values are shown as mean ± standard deviation. MAP = mean arterial pressure, SBP = systolic blood pressure, DBP = diastolic blood pressure, PP = pulse pressure, HR = heart rate, Ds = systolic diameter, ΔD/D = carotid distension, L PWVWH = local pulse wave velocity, RM = reflection magnitude, M BFV = mean blood flow velocity, P BFV = peak blood flow velocity.
p < 0.05.
p < 0.001.
Data based in a sample of 10 volunteers (n = 10).
Data based in a sample of 11 volunteers (n = 11).
General results of selected parameters during the implemented protocol (n = 12).
Hand grip test
Haemodynamic parameters
There was a consistent increase of BP (SBP, DBP, MAP and PP) during both HGT’s in comparison to baseline values, as well as for HR that increased from 63.38 ± 6.62 to 69.29 ± 8.76 bpm for HGT30% and from 61.72 ± 6.43 to 74.80 ± 12.98 bpm for HGT40%. MAP did not fully return to baseline levels over the recovery period, but HR did. Further, blood flow velocity showed a high variability during the tests and no clear trends were observed (see Fig. 5 and Table 2).

Haemodynamic parameters results during each period of all three tests. Black circles represent mean values during a period (indicated below) of each test. Difference from baseline is denoted as significant * (p < 0.05) or highly significant ‡ (p < 0.001).
Carotid (local) parameters
The change in carotid diameter during HGT30% was not statistically significant from baseline, in contrast to the diameter during HGT40%, which was significantly increased. In addition, carotid distension (ΔD/D) showed a remarkable decrease during both HGT’s. During the recovery period, carotid diameter and distension returned to baseline levels.
As is clear from Fig. 6 and Table 2, local PWVWH and RM demonstrated a general high variability, which increased during the testing periods. No clear trends were detectable for these parameters throughout the protocol.
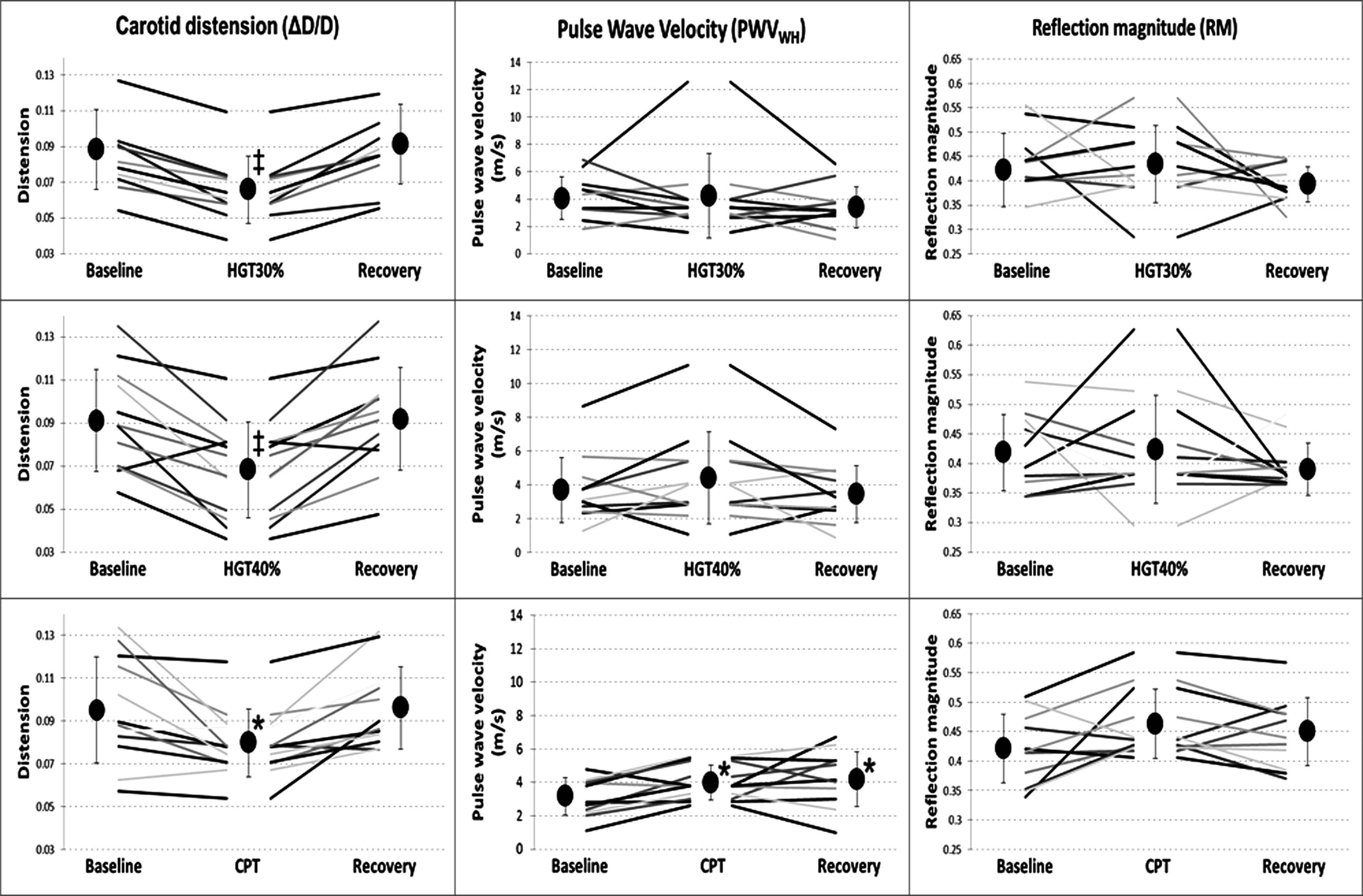
Carotid local parameters results during each period of all three tests. Black circles represent mean values during a period (indicated below) of each test. Difference from baseline is denoted as significant * (p < 0.05) or highly significant ‡ (p < 0.001).
Cold pressor test
Haemodynamic parameters
The highly significant increase in BP during CPT is comparable with the one obtained during both HGT’s while the increase in HR was more modest for CPT. MAP did not fully return to baseline values upon recovery, but HR did. Again, velocity parameters were highly variable, and none of the observed differences were statistically significant. All results are shown in Fig. 5 and Table 2.
Carotid (local) parameters
During CPT, carotid diameter increased while distension decreased, and all returned to baseline values upon recovery. PWVWH and RM exhibited less variability than during HGT. PWVWH increased significantly and did not return to baseline values during recovery while RM presented a borderline significant increase (p = 0.054) during the test period (see Fig. 6 and Table 2).
Discussion
Compatible with previous results,2, 3, 9, 19, 20 handgrip testing (HGT) leads to an increase in both blood pressure (BP) (around +10 mmHg for MAP) and heart rate (HR) (+6–13 beats/minute). Functionally this allows the baroreflex to operate at the prevailing BP evoked by the exercise8 (see Fig. 1C). As illustrated in Fig. 5, the cold pressor test (CPT) led to a similar increase in BP (+11 mmHg), but this increase was not accompanied by a similar increase in HR, as precedent results on CPT have shown.6, 9, 21, 22 This is consistent with the notion, outlined in the introduction, that physical exercise (in this case isometric exercise (HGT)) somewhat “blunts” the baroreflex, suppressing the reduction in HR that one would anticipate in case of active pressure control. Moreover, prior experimentation has verified that the arterial baroreflex is reset from rest to exercise in direct relation to the work intensity.8 This plausibly explains the difference in HR during HGT30% (lower exercise intensity, lower HR) and HGT40% (higher exercise intensity, higher HR). Additionally, baroreflex resetting has been observed at the onset of isometric exercise.23,24 Baroreflex resetting also occurs during CPT though only about 120 s after the onset of the cold stimulus.25 This is consistent with our data, demonstrating that the HR was still under baroreflex control (see Fig. 5 and Table 2).
Similarly to BP trends during all tests, carotid diameter showed an increase even if it was less prominent. Due to a higher distending pressure, an increase in diameter of this elastic artery is slightly blunted by the increase in muscular tone which may explain why the increase in diameter was smaller than the increase in BP. There was a remarkable decrease in carotid distension during all tests albeit trends were different. This can be explained by the increase in muscular tone, operating blood pressure, functional stiffness, and the (inverse) influence of HR.19 Previous studies showed similar outcomes during HGT26 and CPT.21 During recovery, BP did not return to baseline values, while HR, diameter and distension did (see Figs. 5 and 6 and Table 2).
In Fig. 5, additional parameters extracted from US images as mean blood flow velocity and peak blood flow velocity can be observed. However, these results were affected by high variability precluding any further attempts to extract useful information from them.
Results for local PWVWH are presented in Fig. 6 and high variability is clearly a major issue. The same method was used previously to assess PWVWH during resting conditions (∼baseline) in 40 consecutive samples from the Asklepios study.27 They found a PWVWH of 6.00 ± 1.93 m/s28 while the baseline value we obtained was of 3.64 ± 1.53 m/s (mean value of the three baseline periods). As can be expected for a younger population, our local PWVWH value was lower. In another study, a homogeneous population of 22 healthy male volunteers was recruited from the military academy (mean age 21.7 ± 1.4 years, range 19–24 years) to calculate the local PWVWH during baseline (4.28 ± 1.00 m/s) and CPT (5.58 ± 1.68 m/s).21 Although the absolute values in their study are somewhat higher both at baseline and during CPT than the values we calculated, the relative increase of 30% is close to the increase of 26% obtained by us. The differences in absolute value may be due to a difference in methodology: they used pressure curves instead of diameter distension curves for calculating the local PWVWH.
Finally, the RM was calculated. As values rely on local PWVWH for their calculation, also these data showed a high variability (Fig. 6). The data suggest a (borderline) higher RM value during CPT (p = 0.054) possibly as a consequence of an increase in downstream resistance.29 Note, however, that the methodology used to calculate the local PWVWH and the RM might be prone to error. In a recent study, it was demonstrated that although reflection-free periods will emerge as straight sections in single point loop methods as the LnDU loop method, the opposite is not necessarily true: a straight section in the loop does not necessarily mean that wave reflections are absent during that part of the cardiac cycle,30 corrupting the pulse wave velocity estimations in general.28
To the best of our knowledge, few previous studies presented an analysis of the baroreflex based on both HGT and CPT stressors and none included US measurements. This set up delivered us interesting insights not only in the physiological phenomena but on practical issues in order to improve the acquisition and subsequent analysis. Obviously, the proposed protocol can be easily extended, including a dynamic exercise test and/or head down measurements, using an alternative methodology for calculating the local pulse wave velocity or improving the acquisition to synchronize the haemodynamic parameters with the US imaging for assessing the baroreflex sensitivity and for investigating baroreflex resetting during CPT. Moreover, an interesting addition to the set up would be a neck suction device to expand the comprehension over the reset phenomena during different stress stimuli.
Limitations
The sample size was enough to conduct a feasibility study but a larger sample is necessary to draw any meaningful physiological conclusions. Additionally, it was assumed that all volunteers were healthy subjects at the moment of the protocol and a previous screening was not performed.
The study tests were not randomized. Therefore, even if baseline values were in general obtained between tests, it cannot be excluded that repetitive application of the stressors may influence the individual response.
During the protocol, respiratory changes were not tracked and US signals were not acquired continuously neither simultaneously, possibly introducing inaccuracies to calculate local PWVWH and RM. US images could be affected by movement artefacts due to the effort of the volunteers during the tests. This problem was mitigated by the use of a holder but not completely eliminated, causing problems especially for Doppler measurements (velocity parameters).
Conclusions
It is feasible to non-invasively monitor the carotid haemodynamic response to a sympathetic nervous system stimulus using carotid ultrasound, although the variability, especially on the flow-related parameters, is high. A further analysis showed supporting evidence of specific physiological response of the autonomous cardiovascular system to regulate adequately each stress stimulus, suggesting the possibility of assessment of several subsystems involved.
Conflict of interest
There is no conflict of interest related to this paper.
References
Cite this article
TY - JOUR AU - F.J. Londono AU - T.S. Klopmeijer AU - D. Georgakopoulos AU - E.G. Lovett AU - L. Van Bortel AU - P. Segers PY - 2014 DA - 2014/09/20 TI - Carotid haemodynamics during sympathetic nervous system stimulation via handgrip and cold pressor testing in young healthy subjects: A feasibility study JO - Artery Research SP - 178 EP - 188 VL - 8 IS - 4 SN - 1876-4401 UR - https://doi.org/10.1016/j.artres.2014.08.001 DO - 10.1016/j.artres.2014.08.001 ID - Londono2014 ER -